1.4 Why study biology at the molecular level?
There is a molecular basis to life. That is, all biological function depends on events that occur at the molecular level. We now understand that these events are coordinated by (often) complex molecular machinery even if we do not fully understand every aspect of their function. These complex molecular machines are comprised of large molecules or clusters of molecules that include proteins, nucleic acids such as deoxyribonucleic acid (DNA), carbohydrates and lipids.
For example, we now understand the molecular basis of inheritance – that DNA, ribonucleic acid (RNA) and the genetic code transmit hereditary information from parents to offspring. We understand how functional gene products (e.g. proteins) work. That is, we are able to relate the complex and varied structure of proteins to their functional roles. We have developed techniques to map the interactions of these molecules, quantitate their abundance, determine their localisation within the cell and study the processes they mediate.
This molecular revolution began in earnest in the 1950s following key scientific breakthroughs that linked our understanding of inheritance to the internal molecular machinery of cells. It has transformed every aspect of biology. Ecologists can now track organisms using their DNA (see Monitoring the platypus with environmental DNA), evolutionary relationships can be accurately mapped using molecular data, taxonomy is resolved by molecular data, understanding the molecular basis of disease has revolutionised diagnosis and treatments, and molecular understanding has improved agriculture and land management.
If one visits most modern biology laboratories regardless of their discipline, whether it is biomedicine, agricultural biology, zoology, microbiology or botany, they tend to appear alike. This is because there is an incredible similarity and overlap in the technical approaches used, regardless of the biological system being studied. Any differences you do notice will likely be due to the underlying biological system under investigation. For example, a microbiology laboratory may use agar plates upon which to culture microorganisms or a plant biology laboratory may use a greenhouse to grow the plants under study. However, even laboratories that study disparate systems may use common approaches such as culturing vinegar flies (Drosophila) or zebra fish (both animals are common genetic models for various useful reasons). The actual molecular techniques used are likely to be very similar. It is not uncommon for researchers in biology who are working at the molecular level to never see the organism under investigation! All the experiments are undertaken using material extracted from the organism in question. It is also possible that a biologist working on a specific problem does not even see biological material at all! This is because due to the staggering amount of data available, some investigations can now even be undertaken solely in silico; that is, by analysing online databases.
You will find biochemists and molecular biologists working in all biological disciplines. Even if they describe themselves as a zoologist, botanist or ecologist, they will often have functional literacy, if not proficiency, in biochemical and molecular techniques.
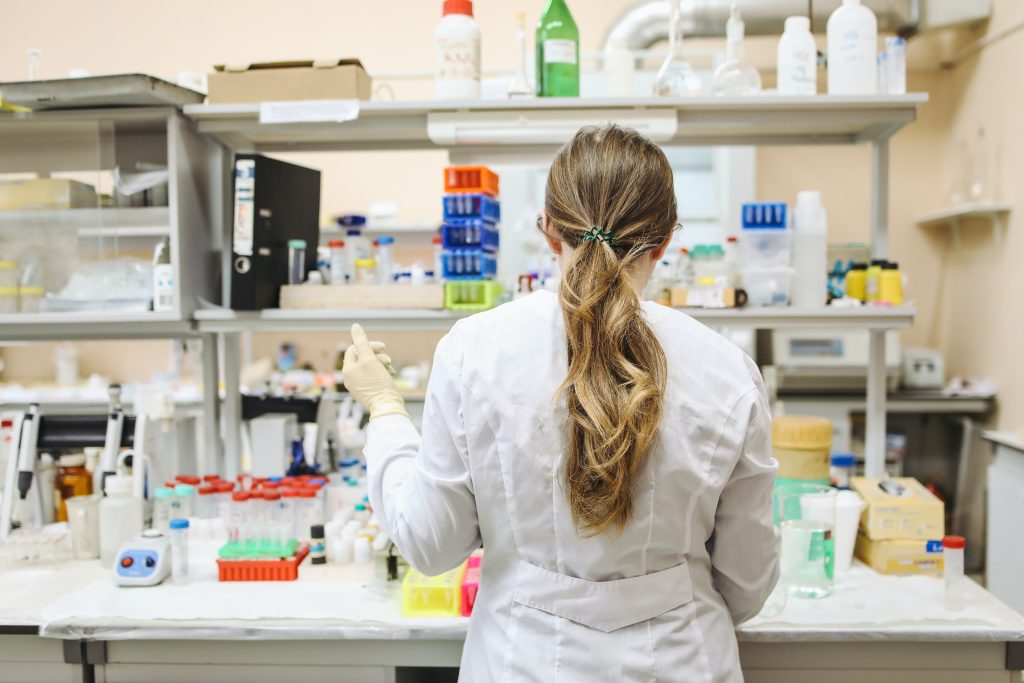
Monitoring the platypus with environmental DNA
All organisms shed DNA into the environment as they lose cells, hair, skin, scales, faeces etc. With recent improvements in technology, it is now possible to collect and sequence this DNA. These trace amounts of DNA, known as environmental DNA (eDNA), can be used to determine which species are present in a particular environment without having to directly observe or capture them. As every organism has a unique DNA sequence, their shed DNA provides a signature that can be used to trace them. For example, from a small sample of water it is possible to obtain a genetic profile of all the organisms present in a waterway.
Australia and New Zealand have been at the forefront in using eDNA to manage both endangered and also invasive species. The iconic Australian animal the platypus is at risk due to habitat loss and drought. eDNA is allowing this notoriously elusive species to be monitored. By understanding its range, environmental scientists are able to make better informed decisions regarding land and waterway management. Since the collection of eDNA is a simple task – a water sample is all that is required – monitoring can be assisted by members of the public. In Australia, one of the world’s largest citizen science projects, The Great Australian Platypus Search, was launched in 2021, to map platypus populations across the state of Victoria.
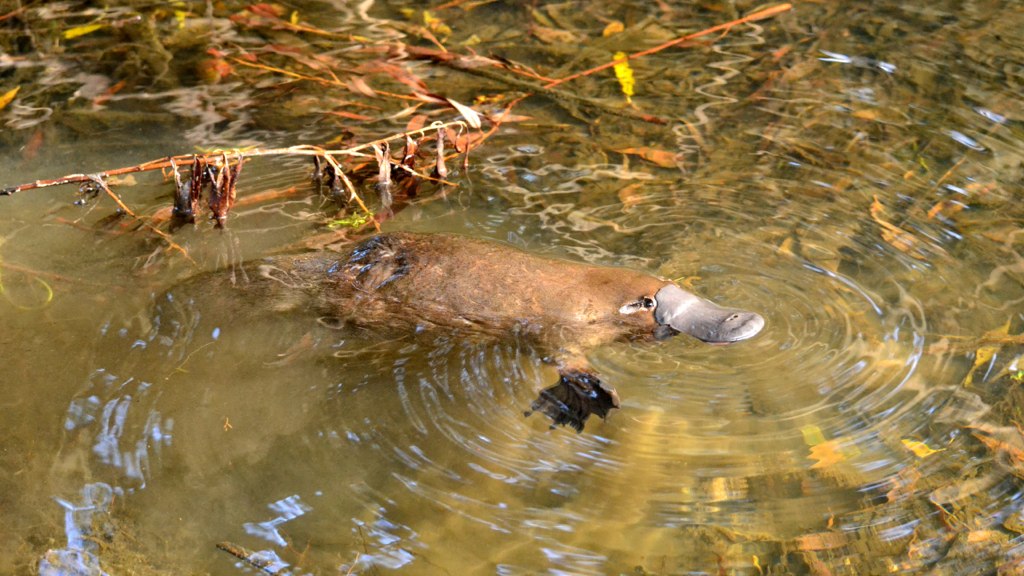
What exactly is analysed in eDNA?
Typically, a gene that is highly conserved and found in all species is used for eDNA analysis. For example, for analysis of the microbiome (all the microbes in an environment) the 16S ribosomal RNA gene is used for identifying species present (all life has ribosomes – see Chapter 3.) For animals, the mitochondrial cytochrome c oxidase I (COI) gene is often used. This gene is useful as it is found in mitochondrial DNA, and mitochondria are found in all eukaryotes. Each cell has many copies of this DNA, so there are more copies of mitochondrial DNA than nuclear DNA. Importantly, there are regions in the sequence of this gene that show enough variability for it to provide a unique signature, allowing it to be used to distinguish individual species.